what type of stresses have the rocks undergo to form the structures
thirteen.1 Stress and Strain
Plate collisions and the accumulated weight of overlying rocks exert forces on rocks at depth. While the size of the strength is important, it also matters whether the force is distributed over a wide region, or tightly focused on a small-scale expanse. The same force will take a greater result when acting over a small area than when interim over a larger area. If you have ever used snowshoes to walk across a snowfall bank without sinking in, you have taken reward of the effects of distributing force (your mass acted upon by gravity) over a wider expanse (the area of your snowshoes rather than the soles of your boots). Stress is force adjusted for the area over which it is distributed. Strain is the change in shape that happens when rocks are deformed by stress.
Types of Stress
Stresses fall into two categories: normal stress acts at right angles to a surface, and shear stress acts parallel to a surface (Effigy 13.2). Normal stress is subdivided into compression, when the stresses are squeezing a rock, and tension, when stress is pulling information technology apart. Rocks undergo compression in regions where plates are colliding, or where they are being buried beneath other rocks. Rocks feel tension where difference is happening, such as when a continent is beginning the rifting process. Shear stress is characteristic of transform plate boundaries, where plates are moving side by side.
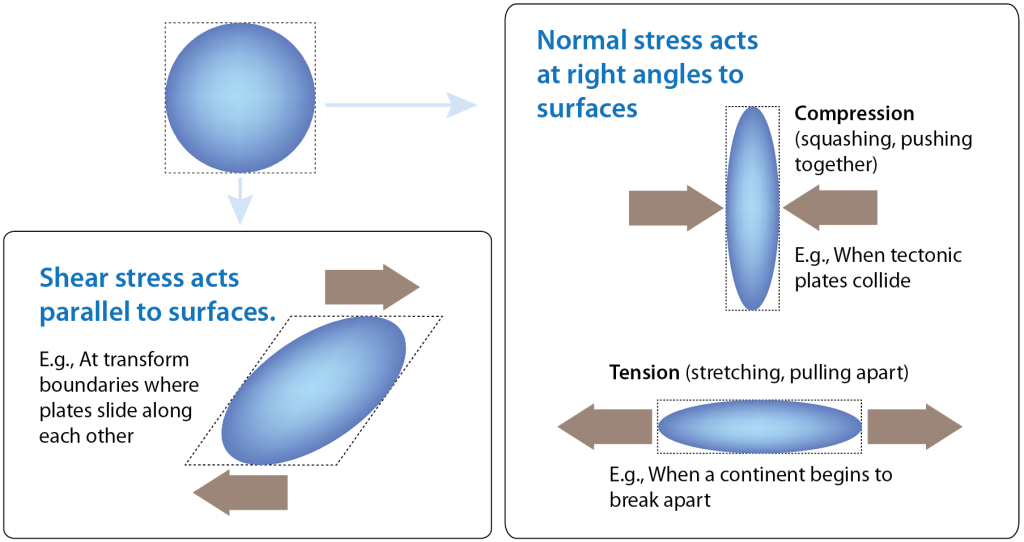
Although Effigy thirteen.2 shows but one set up of stress arrows for each scenario, rocks within the Earth are subject to stress from all directions. The relative size of the stresses in different directions will determine the response of the stone. Consider a deeply buried rock being stretched as a continent breaks apart (Effigy 13.3). It is also existence compressed by the weight of overlying sediments and rocks, but the stress from compression is relatively minor compared to the tension from rifting. The net event of stress acting on the rock will be determined more by the tension from rifting than past the pinch from overlying rocks.

Rocks feel stress from all directions, but it is possible to break down stresses into three directions, just like a graph with x, y, and z axes. In diagrams showing these iii directions, the sizes of arrows representing each direction will indicate the relative size of stresses, as they practice in Figure 13.3. Analyzing stress in this way makes it much easier to describe the stresses operating on a rock, and to empathise what their net effect will be.
Types of Strain
How a rock responds to stress depends on many factors. The "how" is not simply a matter of how much strain a rock will undergo, but what type of strain will occur. Is the deformation permanent or temporary? Does the rock interruption or does it deform without breaking?
Rubberband Strain
Elastic strain is reversible strain. You tin can think of elastic strain as what happens to the elastic waistband of your favourite sweatpants when you lot put them on. The rubberband stretches to let you into your pants, and in one case you're in them, it shrinks to keep them from falling down. When you take the pants off once again, the rubberband goes dorsum to its original shape. Similarly, rocks undergoing elastic strain will snap dorsum to their original shape once the stress is removed. Rocks snapping dorsum to their original shape undergo elastic rebound. Elastic rebound of rocks on a large scale tin have profound consequences, because the energy released causes the Earth to vibrate. We feel those vibrations as earthquakes.
Plastic Strain
If enough stress is applied, the changes that a material undergoes to accommodate the stress will leave it permanently deformed. When the stress is removed, the cloth does not go back to its original shape. The permanent deformation is called plastic strain.
Ductile or Brittle?
Ductile deformation refers to deformation that happens past flowing or stretching. The marble monument in Effigy 13.4 is undergoing ductile deformation as it sags beneath its own weight.
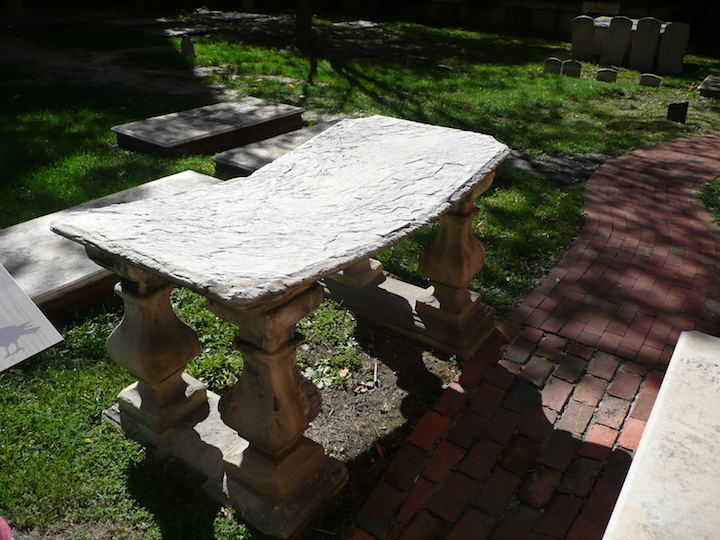
When a textile breaks, it has undergone brittle deformation (Figure 13.five). The stone cylinders in Figure 13.v are part of an experiment to test the strength of the rock. The cylinder on the right looked like the cylinder on the left earlier it was compressed, with force practical to the top and lesser. Strain gauges have been glued on to measure out the amount of deformation lengthwise and across the cylinders.

A cloth can undergo more than i kind of deformation when stress is applied. The barrel-shaped cylinder of potash in Effigy xiii.6 (right) originally looked like the cylinder on the left. The cylinder was compressed, with stress practical from the top and bottom. Initially, information technology underwent ductile deformation and thickened in the centre, creating the barrel shape. But as more stress was applied, the cylinder eventually underwent brittle deformation, resulting in the crack across the centre.

Factors That Determine How A Rock Will Deform
A rock is not limited to exclusively brittle deformation, or exclusively ductile deformation. Even the deformed stone in Figure 13.5, which has clearly undergone breakable deformation, shows a slight curvature on the right side, near the acme. This indicates that a minor amount of ductile deformation occurred before brittle failure.
For a given stone, deformation will exist different depending on the amount of stress applied. Up to a indicate, rocks undergo elastic deformation, and will bound back to their original shape after the stress is removed. If more stress is applied, the stone may deform in a ductile manner. If stress increases further, the rock may fracture. The amount of stress required in each case will depend on the type of rock, as well every bit atmospheric condition such as force per unit area and temperature.
Composition
In general, sedimentary rocks will be more likely to undergo ductile deformation than igneous or metamorphic rocks under the same conditions. Rocks within each group will likewise deform differently.
Boudinage structures (Effigy thirteen.7) highlight the effect of composition on how rocks deform. These structures occur when a stronger rock more decumbent to brittle deformation is surrounded past weaker rocks prone to ductile deformation. The stronger rock will fracture into segments, called boudins, and the weaker rock will flow into the spaces between. In Figure 13.7 (top), the white layer reached the stage of pinching off, just before separating into segments. The surrounding blackness layer flowed in to fill the gap where the pinch was happening. Remarkably, the white layer itself contains a dark layer that has fragmented into boudins. Not all boudins suspension into blocky segments. Some display more ductile deformation (Effigy thirteen.7, bottom).

Temperature and Pressure
At higher temperatures, and nether higher circumscribed pressures, rocks are more likely to undergo ductile deformation. Confining pressure is the stress that a material experiences uniformly from all sides as a result of the weight of textile above and around it. The pressure that a diver feels deep in the ocean is circumscribed pressure due to the weight of water above and around the diver. This kind of confining pressure is chosen hydrostatic pressure. Within Earth, the confining pressure is due to the weight of overlying rocks. Confining pressure due to the weight of rocks is chosen lithostatic pressure.
The rocks in Figures 13.five and 13.half-dozen experienced confining force per unit area from the atmosphere, and temperatures comfortable for the humans working in the lab. Under those conditions the rocks ultimately underwent brittle failure when they were compressed in the lab. Deep within the chaff, the temperatures and confining pressures are far greater. Deep plenty within the chaff, both samples would undergo only ductile deformation if the same amount of stress were applied as in the experiment. The depth at which temperatures and confining pressures are high enough for rocks to get from brittle deformation to ductile deformation is chosen the breakable-ductile transition zone.
The brittle-ductile transition zone occurs between approximately x km and thirty km depth, corresponding to temperatures around 300 ºC and greater. The depth at which temperatures reach 300 ºC at any particular location volition depend on heat flow at that location. In continental crust, rocks at 300 ºC are deeper than in body of water crust. The change in pressure level with depth besides varies, depending on the mass and density of rocks. If depths are measured relative to sea level, the pressure at x km measured below a tall mountain belt will be greater than the pressure at 10 km measured within ocean chaff.
Experiments like those shown in Figures 13.5 and thirteen.half dozen can be used to determine where the brittle-ductile transition zone will be for a particular rock type. Experimenters apply stress to sample of a rock for a range of temperatures and confining pressures. They note the conditions nether which the rock breaks or deforms in a ductile fashion, and plot those on a graph (Figure xiii.8). The results in Figure thirteen.8 are from experiments on limestone. The vertical centrality is pressure. The more pressure, the deeper the rock would have to be within the Globe to experience that pressure. The white line represents the breakable-ductile transition zone. To a higher place the white line are pressures and temperatures nether which the limestone would fracture. Below the white line in the tan expanse are pressures and temperatures where the limestone would deform past flowing. Notice that the higher the temperatures, the less confining pressure is required for ductile deformation.
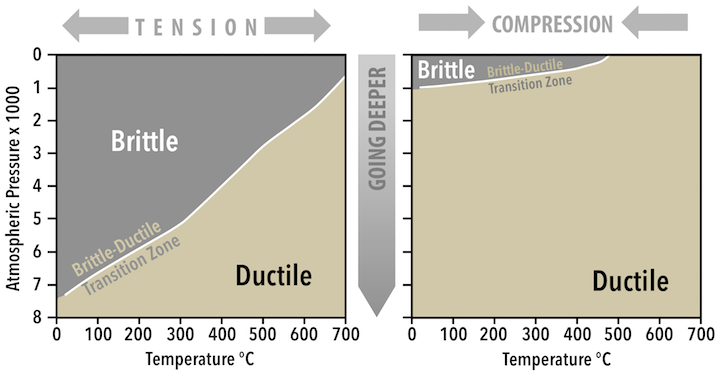
How Stress Is Applied
The limestone experiments were performed by applying stress as tension (Figure xiii.8 left) and once again by applying stress as pinch (right). When tension was applied, temperature and confining pressure had to exist much higher before ductile deformation occurred. Nether compression, ductile deformation was possible with far less confining pressure, and at lower temperatures.
Strain rate, the rate at which deformation occurs, also makes a difference. If stress is applied at a charge per unit that causes rapid deformation, the stone will be more likely to fracture than if deformation happens slowly. The marble slab in Figure xiii.4 is a good case of this. It has sagged rather than broken because the charge per unit of deformation has been very deadening, at millimetres per decade.
Fluids
When rocks are nether pressure level, fluids trapped inside the pore spaces of rocks- the gaps between grains- are also under pressure. Higher confining force per unit area is required for deformation to exist ductile rather than breakable, but pressure from fluids, chosen pore force per unit area, resists the confining pressure level. The result is that the effective confining pressure is lower than it would be without the fluids. Depending on the corporeality of pore pressure, and how close the stone is to the brittle-ductile transition zone, pore force per unit area could crusade brittle failure in a rock that would otherwise undergo ductile deformation.
Stress and Geological Structures
Many unlike geologic structures can form when stress is practical to rocks. Structures form as a result of fracturing, tilting, folding, stretching, and squeezing (Figure 13.nine). Some structures, like the fractures that brand basalt columns (Effigy xiii.9, upper left), happen when rocks shrink due to cooling, but others are a consequence of plate tectonic forces. The types of structures that form depend on the plate tectonic setting and other geological conditions, making them valuable tools for understanding what happened to the rocks. The post-obit sections address the different kinds of structures that form, and what information we can gather from these structures to learn more virtually the tectonic surroundings and regional geology.
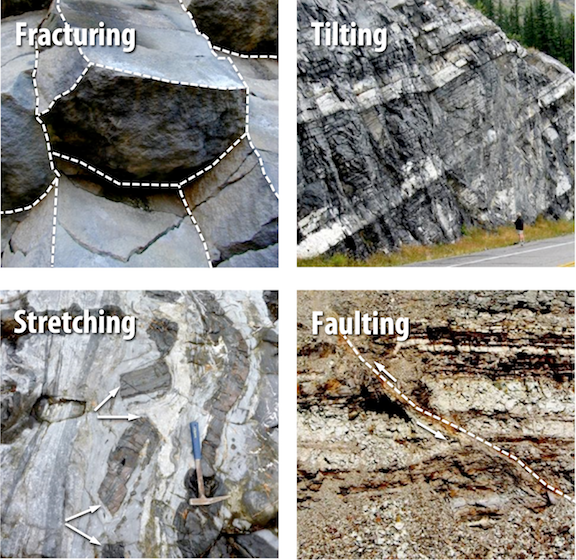
References
Heard, H. C. (1960). Transition from Brittle Fracture to Ductile Flow in Solenhofen Limestone every bit a Function of Temperature, Circumscribed Pressure, and Interstitial Fluid Force per unit area. In D. Griggs & D. Handin (Eds.), Stone Deformation (A Symposium): Geological Club of America Memoir 79 (pp. 193-226). https://doi.org/ten.1130/MEM79
Source: https://openpress.usask.ca/physicalgeology/chapter/13-1-stress-and-strain-2/
0 Response to "what type of stresses have the rocks undergo to form the structures"
Post a Comment